We in the high altitude jet set train extensively for the chance we may one day suddenly lose cabin pressurization and need to immediately don oxygen and execute an emergency descent. But how often does that really happen? For me, the answer is one time in the last thirty-five-plus years: B-707 Depressurization.
— James Albright
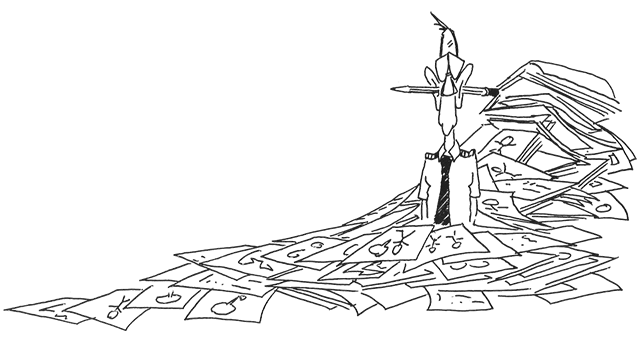
Updated:
2016-07-01
But we are trained for this. When it happens, it will be obvious and we will know what to do. A rapid depressurization has never resulted in the loss on an airplane, at least not that we know of. The real dangers lurks when the loss of pressurization happens gradually. Slow onset hypoxia has killed and you are at risk.
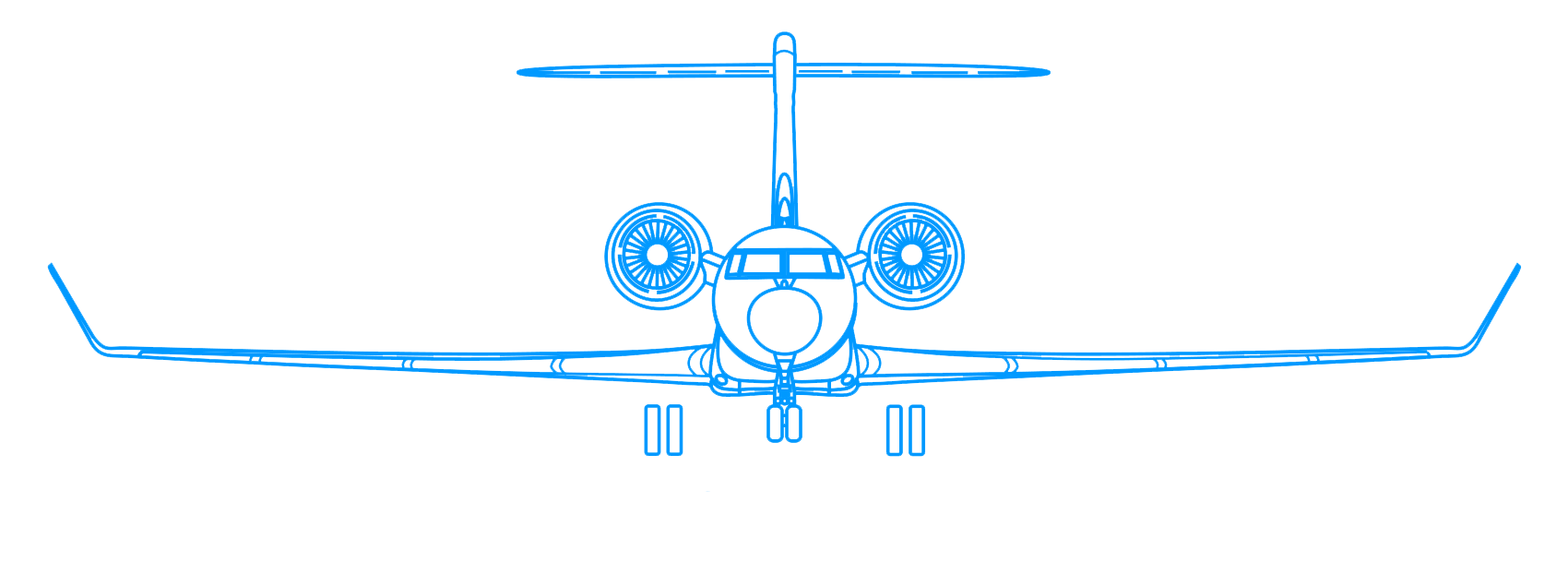
1
A history of losses
There are no documented cases of an airplane lost due to a rapid decompression but there are at least five when the aircraft failed to pressurize properly:
- Beechcraft King Air VH-SKC — The aircraft was unpressurized and the occupants did not receive supplemental oxygen, for undetermined reasons.
- Cessna 421 N48DL — The FAA was negligent enough to permit this airplane to operate at 25,000 feet without oxygen equipment and the pilot was foolish enough to take it to high altitude.
- Helios Airways 522 — A misset pressurization switch left the occupants unconscious until the aircraft ran out of fuel, all aboard were killed.
- Learjet N47BA — The flight crew were unable to receive supplemental oxygen following a loss of cabin pressurization, for undetermined reasons, all aboard were killed.
- Socata TBM 700N C-FBKK — The aircraft apparently lost pressurization, the oxygen system was turned off at the bottle, the aircraft entered a spiral dive.
Pilot appeared to lose useful consciousness 20 minutes after takeoff.
Pilot appeared to lose useful consciousness about 30 minutes after takeoff.
Pilots appeared to lose useful consciousness 13 minutes after takeoff.
Pilots appeared to lose useful consciousness 14 minutes after takeoff.
Pilot appeared to lose useful consciousness 16 minutes after takeoff.
In many of these cases the reason the aircraft did not pressurize was never proven (VH-SKC, N48DL, N37BA, and C-FBKK) or occurred because of an issue that could have been prevented (Helios Airways 522). In a few of the cases the oxygen system was turned off at the source or was never installed in the first place (N48DL and C-FBKK). But in every case the mishap could have been prevented had the pilot adhered to stricter preflight rules and made a conscious effort to check cabin altitude at a few key moments.
More about this below: A Pilot's Defense Against Slow Onset Hypoxia.
2
The circulatory system
Knowing how the oxygen gets from "out there" — be that the ambient air or the oxygen mask — to inside you on its way to the brain can help you understand why slow onset hypoxia is so hard to recognize. Knowing that, you should be motivated to take the preventative steps to follow.
Although the metabolic system is crucial to make everything work, the circulatory system carries the blood, which in turn transports oxygen, carbon dioxide, nutrients, and waste products. This system is comparable to any closed hydraulic system in an airplane, which includes a fluid (blood), a pump (heart), tubing (blood vessels), and the part requiring support of the system (an organ). This system is of most interest to pilots because any change in oxygen levels to the cells immediately changes the performance of many organs, especially the brain; therefore, anatomy will first be described and then the physiology will be explained in more detail.
Source: Basic Flight Physiology, pg. 22
The Heart
- The heart is the pump in this human hydraulic system, which is a closed system with blood flowing from the heart, into arteries, then through capillaries that are spread around the tissues and individual cells. From there, the blood continues into the venous system, through the lungs, and back to the heart.
- Flowing blood is not under the same pressure throughout the system, which is one of the differences compared to an aircraft hydraulic system. This “sinus wave” pressure is why we can feel a pulse. When the heart contracts, the pressure goes up, and we feel that rise. When the heart muscle relaxes, getting ready for the next contraction, there is a decrease in pressure. The heart contracts again before the pressure gets too low, as a result of muscles in the arterial walls.
- Like any other muscle, the heart needs a blood supply, and this is supplied via the coronary arteries. These arteries become blocked in heart disease, leading to heart attacks or poor blood perfusion (ischemia) of the muscle cells. The heart muscle requires oxygen for energy; the heart can fail or become less effective if oxygen does not reach the heart muscle. Total blockage of blood to the heart muscle leads to death of some of the tissues devoid of blood. Dead heart tissue is called a myocardial infarct (myo = muscle, cardia = heart, infarct = dead), or MI. This myocardial infarction, therefore, is another name for heart attack.
- If the heart-muscle fibers do not all contract simultaneously, there are changes in the rhythm and contractility. Fibrillation occurs when the muscle fibers are contracting at different times and not in synchrony, looking like a can of live worms. Rhythm problems also can develop that compromise the efficiency of the heart’s ability to move blood. Any of these situations leads to heart failure.
- The amount of blood that flows out of the heart per minute is dependent on the heart rate (pulse), the volume of blood ejected from the heart, and the force of the contraction. Much of this is controlled by the autonomic nervous system that senses the need for more blood to a specific area of the body. An increase in carbon dioxide and other metabolites also makes the heart go faster to compensate for metabolic needs.
Source: Basic Flight Physiology, pg. 22
The Lungs
- The lung organs exchange oxygen and carbon dioxide from the ambient air drawn into the air sacs (alveoli) with the same gases in the blood (Fig. 2-2). Air enters the lungs through the mouth or nose, into the pharynx, trachea, and then to the main bronchus. This then splits into two bronchi, which further split into thousands of smaller bronchioles and end up in tiny alveoli (tiny rubber-like balloons).
- Capillaries surround these alveoli, and because both walls are so thin, gases can pass back and forth (diffuse), out of and into the air or into the bloodstream. This diffusion and gas exchange at the cell level will be further explained in the section on physiology of respiration.
- Air is pulled into the lungs by decreasing the pressure within the chest cavity. This is accomplished by the diaphragm, a muscular, dome-shaped covering of the lower part of the rib cage, forming a closed container with one opening, the bronchus. When this muscle contracts, it flattens out, creating lower pressure and bringing in air to fill the alveoli. This inhalation is an active event (a muscle contracting), whereas exhaling is passive when the muscular diaphragm relaxes and returns to its dome shape. The muscles between the ribs also supplement this activity.
- This process of breathing is also an autonomic function, controlled by the CNS and feedback data from various organs telling the breathing rate to change. With exercise, both breathing and heart rate are selectively increased to meet demands without the person having to think about it. We can also easily and actively control our breathing rate, whereas it is difficult to actively control heart rate.
Source: Basic Flight Physiology, pg. 24
The Vascular System
- The link between the heart, lungs, brain, and other parts of the body is the blood vessels, and they maintain an uninterrupted blood supply to all tissues of the body. It is a closed system of arteries and veins. Basically, arteries distribute the blood from the heart to various organs, getting smaller (arterioles) as they branch out. The collecting vessels that return blood to the heart are veins; smaller veins are called venules. Very small vessels at the tissue-cell level are called capillaries.
- Pulmonary arteries carry oxygen-poor blood from the heart to the lungs. Pulmonary veins carry oxygen-rich (reoxygenated) blood from the lungs to the heart. Oxygen-rich blood is then pumped through the arteries of the circulatory system to the tissues and individual cells. Arteries are unique among blood vessels in that they have muscle and elastic cells within their walls, allowing them to dilate or constrict by muscle contraction or the inherent elasticity.
- This is an effective way of increasing or decreasing (shunting) blood flow to various parts of the body. For example, during exercise, the leg muscles need more blood, so arteries to these muscles automatically dilate to their full size. Arteries to other parts, such as the digestive tract, are constricted by the wall muscles, shunting blood away from that area and into the legs. The same process happens in control of body temperatures.
- The elasticity of the artery wall helps keep the blood pressure more constant during the period when the heart muscle relaxes between beats (contractions). Like blowing up a balloon, the pressure within the artery resulting from the elasticity enhances and prolongs the blood pressure that is generated by the heart during the relaxed phase.
- Veins are simple tubes without muscles or elastic tissues. Some veins in the arms and legs have one-way valves that prevent blood from flowing backward during the pause or relaxed phase after it has been pumped forward during a heart contraction. This is necessary because blood pressure in the veins is very low, less than 10 millimeters of mercury (mm Hg), as compared to pressure within the major arteries of about 120 mm Hg at the height of a heart contraction. This low venous pressure is inadequate to move blood back up to the heart and needs the help of our “muscle pump”.
- Contracting arm and leg muscles that surround veins compress the vessel’s wall and squeeze the blood back to the heart in only one direction because of the one-way valves. This is often called the “muscle pump.” Such action does not take place when you have been sitting for a long period of time and blood has pooled in the extremities (stagnant hypoxia). Veins carry blood that is deficient in oxygen and high in carbon dioxide.
- Tissues and cells get their oxygen and nutrient supply from blood flowing next to the cells in the smaller capillaries. These very thin-walled tubes allow diffusion of gases through the walls from the blood to the cell and back again.
Source: Basic Flight Physiology, pg. 26
Respiration
- Respiration is the exchange of gases between the body and its tissues and the outside ambient air. The main objective of respiration is to add oxygen and remove carbon dioxide. Part of this activity requires transferring the gases from another gas mixture (the atmosphere) to a liquid (blood). It is more than just dissolving a gas in a liquid.
- Blood is essentially cells and a liquid called serum. About 55 percent of blood is serum, and about 90 percent of serum is water. One of the blood cells is a red blood cell, which physically carries oxygen molecules attached to a chemical on the cell called hemoglobin. The comparison of partial pressures and the percentage of hemoglobin saturation is called the dissociation curve. Inadequate oxygen to the cells (hypoxia) will occur when the saturation goes below 72 percent, where the curve drops off quickly with decreasing oxygen partial pressure.
- The red blood cells transport oxygen through the use of the hemoglobin. Serum can carry only a small amount of oxygen in solution. The difference between the body’s needs and what the blood can carry is made up by the hemoglobin’s ability to carry oxygen. Any change in the hemoglobin, the number of red blood cells, or the diffusion of gases will cause some form of hypoxia; therefore, respiration involves taking in air, transferring it to blood, and then transporting it via arteries to the cells. The whole process is reversed for removing waste gases via the veins.
- Red blood cells are unique in how they carry oxygen. The bright-red color of arterial blood results from the combination of oxygen with hemoglobin. The darker color of venous blood is an indication of hemoglobin that has minimal oxygen. The amount of oxygen carried (or saturated) by the red blood cells is normally (and needs to be) about 95–98 percent.
Source: Basic Flight Physiology, pg. 27
3
The recognition problem
Times of useful consciousness /effective performance times
- Acute altitude hypoxia affects critical organ systems in different manners. The respiratory and cardiovascular systems, which in the healthy individual are reasonably tolerant of moderate deficiencies of oxygen, respond by attempting to increase oxygen delivery, whereas the CNS becomes more or less dysfunctional as a result of oxygen deficiency.
- The cardiovascular response to altitude hypoxia is to increase cardiac output. The volume of oxygen extracted from the systemic circulation (oxygen consumption) is a product of the rate of delivery (cardiac output) and the proportion of oxygen off-loaded (the arteriovenous oxygen difference). As noted earlier, increased oxygen demand such as with exercise is usually met by increasing both cardiac output and oxygen extraction. However, mixed venous saturation can only fall so much. With progressively higher altitudes and falling oxygen tensions, the volume of oxygen that can be extracted from arterial blood becomes progressively limited and an increased cardiac output is required to meet even resting oxygen demand.
Source: Fundamentals of Aerospace Medicine, pg. 33
You can expect an increased heart rate early on.
- The CNS is disproportionately affected by hypoxia. It is the first tissue to malfunction with oxygen deficiency, and the first one to succumb to anoxic conditions. Oxygen consumption by the brain is characterized by its relative consistency; it is at a high level at rest, and does not change significantly in response to states such as exercise. The requirement by the CNS for an unfailing supply of oxygen is perhaps best illustrated by the physiologic lengths to which the organism will go after a traumatic episode, by supplying blood to the brain at the expense of nearly all other tissues.
Source: Fundamentals of Aerospace Medicine, pg. 33
Your brain will continue to function, though not as well, as other things begin to shut down.
- The warning symptoms of hypoxia tend to be subtle, and the symptom onset insidious. Recognition of developing hypoxia is also hampered by the fact that the cognitively impaired individual has difficulty recognizing his or her own cognitive impairment; an example is the intoxicated driver who is convinced he is in full control of his vehicle. Therefore, considerable attention should be devoted to acquainting the aviator with the early warning signs of hypoxia.
- Signs of CNS dysfunction, some of which may also be noted as symptoms by the individual, include confusion; behavioral changes such as excitement or belligerence; loss of coordination; and eventually unconsciousness. Symptoms of hypoxia may include dyspnea, headache, lassitude, drowsiness, euphoria, and blurred or tunnel vision. Symptoms due to hypocarbia, such as circumoral or acral paresthesias, commonly accompany hypoxic symptoms and are often the first symptoms noted by the aviator.
- Below 3,048 m (10,000 ft), few normal individuals notice hypoxic symptoms while at rest, although measurable deficiencies in color and night vision exist. Depending on the individual, scotopic visual function may be reduced by approximately 10% at 1,524 m (5,000 ft), with a 28% reduction at 10,000 ft. From altitudes of 10,000 ft to approximately 15,000 ft, cardiorespiratory compensation will allow the unacclimatized individual to function, typically for an indefinite period of time. Nonetheless, some degree of impairment is the rule with decreased alertness and impaired judgment and coordination. Above approximately 15,000 ft, the unacclimatized individual will usually become severely impaired usually within a matter of minutes or even seconds, depending on the altitude. However, it should be noted that individual tolerances vary markedly. The length of time an individual is able to perform useful flying duties is known as the effective performance time (EPT), or time of useful consciousness (TUC). This does not reflect the onset of unconsciousness per se—at 18,000 ft, for instance, the individual might not reach such a state—but rather the period of time beyond which the aviator would be unlikely to take corrective or protective action. Typical EPT values for resting individuals are given in [the table].
- Note that at higher altitudes, EPT values are considerably shorter than the period of time the average individual could breath-hold. Although at altitude mixed venous oxygen tension falls below its typical resting level of 40 mm Hg, at extreme altitude alveolar oxygen tension will fall lower still, resulting in a net diffusion of oxygen out of the pulmonary capillary. Therefore, EPT at that altitude is a function of circulation time. Exercise of even modest levels shortens the EPT because decreased circulation time and increased peripheral demand result in a faster loss of oxygen.
Altitude | Effective Performance Time | |
Meters | Feet | |
5,500 | 18,000 | 20 - 30 min |
6,700 | 22,000 | 10 min |
7,600 | 25,000 | 3 - 5 min |
8,500 | 28,000 | 2.5 - 3 min |
9,100 | 30,000 | 1 -2 min |
Source: Fundamentals of Aerospace Medicine, pg. 33
Your time of useful consciousness (TUC) following a rapid decompression is well documented, though it is doubtful the average pilot can approach these numbers. (These times were based on Air Force pilots in their twenties, sitting in altitude chambers, fully prepared for the decompression. If you are not as fit as they were, not as young, and not as prepared, your times are going to be worse.)
- Rapid loss of aircraft pressurization dramatically reduces TUC. As a general rule, it can be assumed that the TUC following decompression to altitudes between 25,000 ft and 43,000 ft will be reduced by 50 percent. Above 43,000 ft, the TUC is reduced to the time it takes for the blood to circulate from the lung to the brain, plus any reserve oxygen stored in the brain. This is approximately 9–12 s from the start of a rapid decompression to the loss of functional capability. In some cases, hypoxia exposure can lead to a shock type reaction that could further reduce the TUC.
Source: AC 61-107B, ¶2-7.e.(3)(a)
There is a distinct advantage to losing your cabin pressurization suddenly: you will know about it and you will know what to do about. You've trained your entire high altitude pilot career for this. But what happens when you lose pressurization gradually?
Stages of Hypoxia
Now what about for those times you lose pressurization gradually, or you somehow climb into the thinner air without it?
Stage | Altitude in feet | Arterial oxygen saturation (percent) | |
Breathing air | Breathing 100% Oxygen | ||
Indifferent | 0 - 10,000 | 33,000 - 39,000 | 95 - 90 |
Compensatory | 10,000 - 15,000 | 39,000 - 42,200 | 90 - 80 |
Disturbance | 15,000 - 20,000 | 42,200 - 45,200 | 80 - 70 |
Critical | 20,000 - 23,000 | 45,200 - 46,800 | 70 - 60 |
Table 1-7: Stages of Hypoxia
- Many observations have been made on the subjective and objective symptoms of hypoxia. A detailed analysis of progressive functional impairment indicates that the effects of hypoxia fall into four stages. Table 1-7 summarizes the stages of hypoxia in relation to the altitude of occurrence, breathing air or breathing 100 percent oxygen, and the arterial oxygen saturation.
- Indifferent Stage. There is no observed impairment. The only adverse effect is on dark adaptation, emphasizing the need for oxygen use from the ground up during night flights.
Source: U.S. Naval Flight Surgeons Manual, pg. 1-20
Most pressurized aircraft have a maximum cabin altitude of between 6,000 and 8,000 feet. Under normal conditions, dark adaptation to your vision should be the only impairment.
- Compensatory State. The physiological adjustments which occur in the respiratory and circulatory systems are adequate to provide defense against the effects of hypoxia. Factors such as environmental stress or prolonged exercise can produce certain decompensations. In general, in this stage there is an increase in pulse rate, respiratory minute volume, systolic blood pressure, and cardiac output. There is also an increase in fatigue, irritability, and headache, and a decrease in judgment. The individual has difficulty with simple tests requiring mental alertness or moderate muscular coordination.
Source: U.S. Naval Flight Surgeons Manual, pg. 1-20
While everyone reacts differently, most pilots begin to notice their symptoms around 18,000 feet. Unfortunately, these pilots may have suffered impairments to judgment prior to recognizing their usual symptoms.
- Disturbance Stage. In this stage, physiologic responses are inadequate to compensate for the oxygen deficiency, and hypoxia is evident. Subjective symptoms may include headache, fatigue, lassitude, somnolence, dizziness, “air-hunger“, and euphoria. At 20,000 feet, the period of useful consciousness is 15 to 20 minutes. In some cases, there are no subjective symptoms noticeable up to the time of unconsciousness. Objective findings include:
- Special Senses. Peripheral and central vision are impaired and visual acuity is diminished. There is weakness and incoordination of the extraocular muscles and reduced range of accommodation. Touch and pain sense are lost. Hearing is one of the last senses to be affected.
- Mental Processes. The most striking symptoms of oxygen deprivation at these altitudes are classed as psychological. These are the ones which make the problem of corrective action so difficult. Intellectual impairment occurs early, and the pilot has difficulty recognizing an emergency situation unless he is widely experienced with hypoxia and has been very highly trained. Thinking is slow; memory is faulty; and judgment is poor.
- Personality Traits. In this state of mental disturbance, there may be a release of basic personality traits and emotions. Euphoria, elation, moroseness, pugnaciousness, and gross overconfidence may be manifest. The behavior may appear very similar to that noted in alcoholic intoxication.
- Psychomotor Functions. Muscular coordination is reduced and the performance of fine or delicate muscular movements may be impossible. As a result, there is poor handwriting, stammering, and poor coordination in flying. Hyperventilation is noted and cyanosis occurs, most noticeable in the nail beds and lips.
- Critical Stage. In this stage of acute hypoxia, there is almost complete mental and physical incapacitation, resulting in rapid loss of consciousness, convulsions, and finally in failure of respiration and death.
An important factor in the sequence cited above is the gradual ascent to altitude where the individual can come to equilibrium with the gaseous environment, and physiological adjustments have sufficient time to come into play. This occurs in military aviation only in cases where the aviator is unaware that his oxygen is disconnected or in cases where leaks occur in the oxygen system, causing gradual dilution of the oxygen with cabin air.
Source: U.S. Naval Flight Surgeons Manual, pg. 1-20
- No matter what the reason for oxygen not getting to the cell or being used in its metabolism, the lack of oxygen (hypoxia) results in a variety of subtle and not-so-subtle symptoms: any single or concurrent combination of several situations causing some degree of incapacitation. The danger of hypoxia is that the pilot is probably unsuspecting that he/she is hypoxic.
- The key to flying safe at altitude is to recognize:
- The conditions under which you could be hypoxic.
- The physical and mental symptoms that indicate you are hypoxic.
- When a crewmate is susceptible to hypoxia in those conditions.
- Because the nervous system tissues have a heavy requirement for oxygen, especially the brain (and eyes), most hypoxic symptoms are directly or indirectly related to the nervous system. If hypoxia is prolonged, serious problems develop with ultimate death. In extreme cases (prior to death), some brain cells are actually killed, and they cannot be regenerated. It bears repeating. The single most dangerous characteristic of hypoxia is that if the crewmember is hypoxic and engrossed in flight duties, the pilot might not even notice the impairment. Only through education, continued awareness, and actual exposure to hypoxia in controlled conditions (as in an altitude chamber) can the pilot truly respect this insidious hazard.
- Because hypoxia can and often does develop gradually, the pilot must recognize its various stages, allowing some degree of anticipation if symptoms in the early stages are identified. The earlier that hypoxia can be recognized, the sooner that corrective action can be taken before the pilot becomes unable to act appropriately. Keep in mind that although altitude is the common denominator in being hypoxic, the health of the pilot can affect his tolerance. Other abuses, such as smoking, alcohol, and stress, can reduce this tolerance in the otherwise healthy pilot; therefore, hypoxia can be very unpredictable. Saying you had no problem at 10,000 feet last week does not mean you will safely function today at the same altitude.
Source: Basic Flight Physiology, pg. 54
Symptoms of Hypoxia
- The following are some of the more common symptoms experienced by pilots with hypoxia (not necessarily in any order of severity):
- Change in peripheral vision, even noting “tunnel vision.”
- Visual acuity impairment, images slightly blurred, can’t focus.
- Difficulty in visual accommodation, focusing from near to distant and back.
- Weakness in muscles, more difficult to change the airplane seat.
- Feeling very tired and fatigued, sleepy for no reason (not boredom).
- Sense of touch is diminished, the controls feel different.
- Sense of pain is diminished (the aching sprained ankle is better).
- Headache, especially if hypoxic for a long period (2+ hours).
- Lightheaded and mild dizziness, reacting poorly in tight turns.
- Tingling in fingers and toes.
- Muscular coordination decreased, sloppy at controls.
- Stammering, can’t get the right words out to ATC.
- Cyanosis, bluish lips and fingernails (notice in your crewmate).
- Impaired judgment, doing dumb things, slow thinking.
- Loss of self-criticism, no longer care if you’re a great pilot.
- Overconfidence, “No problem!”, taking risks not normally acceptable to that individual.
- Overly aggressive, too much in charge, challenging ATC.
- Depression, small irritants become perceived as major problems.
- Altered respiration, breathing faster, shallower.
- Reaction time decreased, you’ve lost your touch.
- Greatly reduced color discrimination and night vision (even at 5,000 feet).
- Euphoria, you settle for less, who cares?Difficulty in visual accommodation, focusing from near to distant and back.
- In summary, the signs and symptoms of hypoxia are many and varied, and they differ from individual to individual and are unpredictable at any given time and altitude. Be aware, be suspicious, and watch your crewmates. Hypoxia is not a black or white condition. It is insidious, with various levels of impairment.
Source: Basic Flight Physiology, pg. 56
4
A pilot's defense against slow onset hypoxia
- Address pressurization system problems immediately, thoroughly, and by the book — Small pressure vessel leaks get larger with time.
- Oxygen supply and delivery equipment must be pre-flighted prior to every flight — Oxygen systems tend to be sturdy until they aren't. A two dollar Schraeder valve, for example, can last for years and then simply quit. A full oxygen system can empty itself overnight. The only way to be sure is to check.
- Check cabin altitude passing 10,000 feet, 18,000 feet, and every hour after that, you should not rely on cockpit warning systems — There are times the warning systems either malfunction are designed in such a way they can be missed.
- Understand your airplane's normal cabin pressure climb schedule.
- If your cabin normally climbs at 500 fpm, you should not see more than 5,000 feet cabin altitude after 10 minutes. If you do, you have a problem.
- If you are passing 10,000 feet aircraft altitude about five minutes after takeoff and your pressurization system climbs at 500 fpm, your cabin should only be at 2,500 feet. You should become familiar with where your cabin is at this point in the flight so as to know when things are not as they should be.
- If your aircraft's maximum cabin altitude is 6,000 feet for normal departure and destination conditions (you are not operating at high elevation airports), a cabin altitude of 7,000 feet means you have a problem.
- When troubleshooting a pressurization problem, don oxygen — Donning oxygen quickly will not only improve your mental capabilities, it can keep you in the game if things go south quickly or insidiously. You can get so wrapped up in troubleshooting you may not have the judgment to think about oxygen before it is too late.
- When in doubt, don your oxygen mask and descend — You can replenish the oxygen in your blood for very poor (63% saturation) to very good (99%) in less than a minute. But you have to be familiar with your mask so that donning it becomes second nature. Pilots who have never operate their aircraft's quick donning oxygen mask are unlikely to succeed if they are already hypoxic.
Case Study: Helios Airways 522.
Case Study: Learjet N47BA.
Case Study: Socata TBM 700N C-FBKK.
Case Study: Beechcraft King Air VH-SKC.
Case Study: Helios Airways 522.
5
Treatment steps
There are two problems with waiting until you clearly recognize you are suffering from hypoxia. First, your physical reactions will be impaired by poor coordination and possibly by weakened muscles. Second, your brain may exhibit a lackadaisical tendency that makes it no longer care that your recover or not. There is a way to combat both problems.
If there is any suspicion of hypoxia in yourself or a crewmate, the immediate use of 100 percent oxygen is essential. Aside from the rare occurrence of oxygen toxicity, you cannot hurt anyone by giving them oxygen. If hypoxia is occurring, then there will be almost immediate response. If there isn’t this immediate improvement, you must think of other causes for the symptoms. If supplemental oxygen is not available, then getting the airplane or cabin altitude below 10,000 feet is mandatory. Hypoxia is a valid reason to declare an emergency to ATC.
Source: Basic Flight Physiology, pg. 63
Notes From an Altitude Chamber Operator
- Most aircraft are designed with a 8,000 foot cabin altitude where you are down to about 92% saturation levels.
- Of 2800 pilots through his chamber, there were no differences in gender or fitness, but older pilots seem to recognize their symptoms better
- 90% of pilots recognize their first symptoms at 18,000 feet
- Average time to recovery from 63% to 99% saturation level is less than 1 minute after going on 100% oxygen
- Should cross check after 1 minute with the other pilot, "are you okay?"
- Average time to losing consciousness in a climb at 1,500 fpm is 9.2 minutes
Source: Dr. Paul W. Buza, Medical Director, Southern AeroMedical Institute, Florida, 321-676-3200
The problem is that judgment has been impaired long before this point and the pilot may not have the wherewithal to take corrective actions unless it is an automatic, practiced response.
6
A note about oxygen masks
Quick donning oxygen masks are generally designed to go from a stowed position to your head in five seconds or less, even if you are wearing eye glasses. If you really need the mask, will you be able to don your mask that quickly? A few pointers:
- If you are dealing with a rapid depressurization the air will have been sucked out of your chest and the oxygen saturation level in your blood will be on the way down. Simply pull the mask out of its container and bring it to your face. Take a few breaths to calm down and replenish the oxygen in your blood. Make sure the mask is always stowed in the 100% position.
- You should be used to using the mask at high altitudes and should be reasonably proficient at donning the mask quickly. You aren't? You really ought to practice.
- Once you've got those first few breaths in, it is time to wear the mask. Every cockpit is different and you should know how to configure your communications panel properly.
- There is a misguided technique of placing the mask on one's lap in lieu of wearing it. If you have a rapid depressurization the violence of the air movement could rip that mask right out of its socket. It is best to wear the mask; but if you aren't going to do that, leave it stowed.
References
(Source material)
Advisory Circular 61-107B, Aircraft Operations at Altitudes Above 25,000 Feet Mean Sea Level or Mach Numbers Greater than .75, Change 1, 9/9/15, U.S. Department of Transportation
Aeronautical Information Manual
Australian Transport Safety Bureau, Investigation Report, 200003771, Pilot and Passenger Incapacitation, Beech Super King Air 200 VH-SKC, Wernadinga Station, Qld, 4 September 2000, Department of Transportation and Regional Services,
Davis, Jeffery R., etal., "Fundamentals of Aerospace Medicine," Fourth Edition, Lippincott, Williams & Wilkins, 2008
NTSB Aviation Accident & Synopsis Narrative, N48DL, ERA12LA290
Reinhart, Richard, M.D., "Basic Flight Physiology," Third Edition, McGraw Hill, 2008.
U.S. Naval Flight Surgeon's Manual, Third Edition, 1991, Naval Aerospace Medical Institute