We've been teaching stall recoveries all wrong for years and we've killed some people as a result. Finally, after the needless loss of all aboard Colgan Air 3407, the collective aviation community has seen the light.
— James Albright
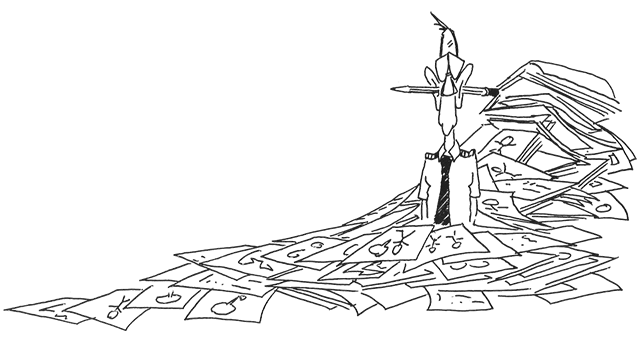
Updated:
2015-03-18
There used to be a stall recovery procedure in the G450 manual, G450 AOM 06-03-30: "Recovery from stalls accompanied by the stick pusher is accomplished by smoothly advancing power and rolling wings level. As airspeed increases, pitch is increased to reduce altitude loss. Excessive or sudden pitch changes should be avoided to prevent entering a secondary stall accompanied by the stick pusher." The diagram above was from that manual, and it was wrong, wrong, wrong.
Whenever you took a check ride in the past, the objective was to minimize the amount of altitude loss. Once again: wrong, wrong, wrong. What should the objective be? It should be to get the wing out of the stall as soon as possible. If you have altitude, use it!
Compounding simulator training that teaches a focus on altitude preservation, most big airplane pilots have never actually stalled a big airplane. Yes the simulator is good, but it isn't that good. Why is this important? A swept wing, T-tailed aircraft stalls differently than most aircraft used for stall training. Where a Cessna 152 or Piper PA-28 pitch down during a stall, most swept wing aircraft actually pitch up.
1 — How wing sweep affects stall onset
2 — How wing shape affects stall warning and roll control
3 — How tail design affects pitch control and stall
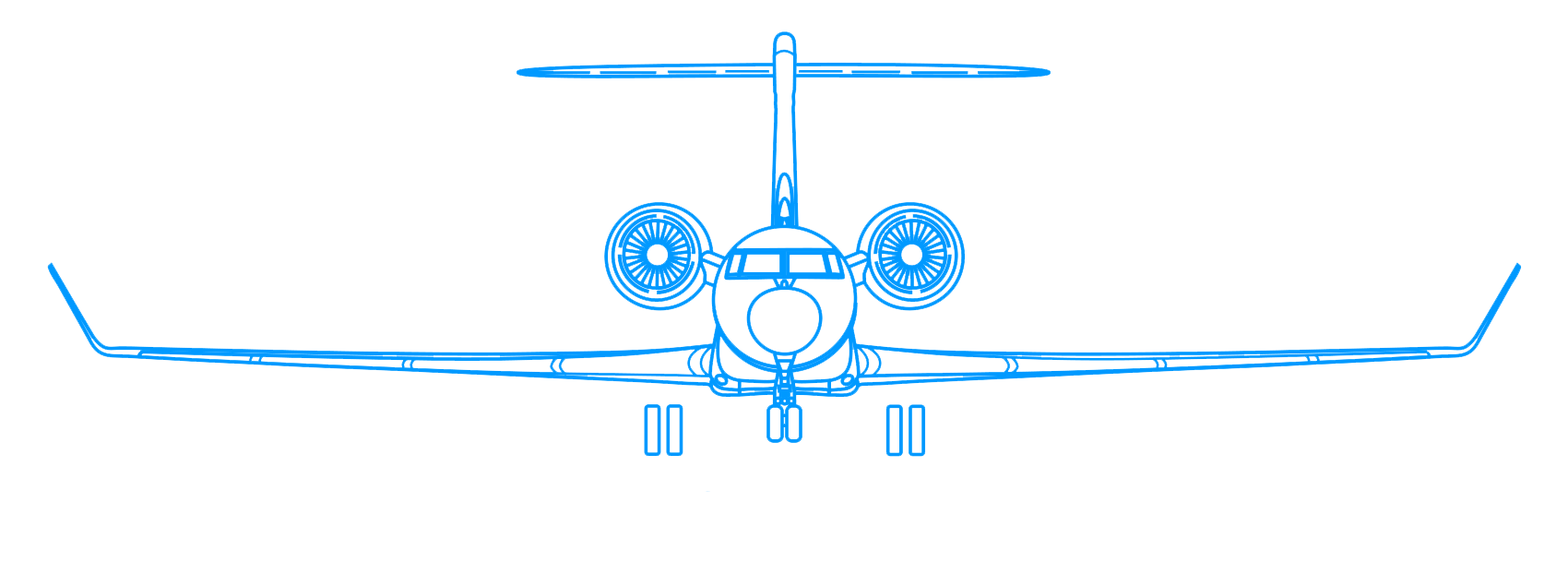
1
How wing sweep affects stall onset
Each wing type stall differently . . .
Throughout the history of aviation, the slow speed region of flight has been the most hazardous. The reasons for this are quite different for propeller aircraft and for turbojet aircraft. Examination of the lift coefficient curves for each type of aircraft will help explain some of these differences. [The figure] shows the curves for a straight wing, propeller driven aircraft and a swept wing, jet aircraft. Note: the type of propulsion doesn't matter to the curves, it is the wing sweep.
Three differences can be seen from these curves:
- The straight wing aircraft has a higher value of CL. Recall the basic lift equation:
- The swept wing aircraft must fly at a higher AOA to achieve maximum lift.
- There is a sudden reduction of CL for the straight wing aircraft at stall, but not for the swept wing aircraft.
It can be seen that if the lift equals the aircraft weight, stall speed will be a minimum when the values of the lift coefficient is a maximum (CL(MAX)), and that the higher the value of CL(MAX), the lower the stall speed will be. This, of course, means that the straight wing aircraft has a lower VS for the same weight and wing area.
Source: Dole, pgs. 125-126
The straight wing aircraft will reach its maximum lift at an earlier angle of attack, but the amount of lift produced by the wing drops sharply with any further increases in AOA.
The swept wing aircraft requires a higher angle of attack to reach its maximum lift and further increases in AOA only result in smaller decreases in lift production.
2
How wing shape affects stall warning and roll control
You might not have any natural warning at all . . .
Stall of a wing will occur where the ratio of the local lift coefficient Cl to the wing lift coefficient CL is highest. . . . The straight wing is usually rectangular, or it has a slight taper. The wing area near the wing tip is large compared to the lift in that area, and the wing loading is, therefore, low. . . . The maximum wing loading is at the wing root. Stall will, therefore, start at the wing root and then spread outward and forward as shown in [the figure].
Swept wings have more taper, with the extreme being the delta wing with a taper ratio of zero. The delta wing's area at the tip is very small, and the wing loading is very high. Stall starts where the wing loading is highest, so the stall starts from the wing tip and progresses inboard and forward.
Straight wing aircraft, with stall starting at the roots, seem to have advantages over swept wing aircraft, which stall at the tips. First, there is more adequate stall warning, caused by separated air buffeting the fuselage. Second, the ailerons of the straight wing aircraft are not enveloped in the stalled air until the stall has progressed outward from the wing roots, compared to the relatively early envelopment from tip stalling.
Source: Dole, pg. 126
A straight wing airplane gives you an early warning of a stall with buffeting near the fuselage; you have full use of your ailerons until the stall has progressed well beyond the earliest warning.
A swept wing airplane gives only the slightest warning at the earliest stages of the stall, the buffet will be far from the fuselage. As the stall progresses, the ailerons quickly become impacted by the turbulent air at the wing tips.
3
How tail design affects pitch control and stall
Low-tailed aircraft
The forces in the pitching plane are shown [in the figure]. Assume the aircraft is trimmed in straight and level flight and that the aircraft's CG is forward of the AC (as shown). A downward balancing force on the tail is required. In case of an aft CG location, an upward force on the tail is required.
If the speed is reduced, a gentle up deflection of the elevator is required to maintain altitude, and the downward load on the tail is increased. The static stability of the aircraft causes a nose-down pitching tendency, which has to be resisted by further up elevator to keep the nose from dropping. The low set tail soon becomes engulfed in the turbulent, low energy air from the wing wake. This reduces the efficiency of the tail.
Source: Dole, pg. 258
The tail becomes less effective as the aircraft begins to stall.
At the stall, two distinct things happen. The airplane responds to the traditional nose-down pitching tendency at the stall, and the whole airplane responds with a nose-down pitch. Second, at the moment of stall, the wing wake passes straight aft and goes above the low set tail. This leaves the tail in undisturbed, high energy air, and it now is at a high positive AOA, causing an upward lift on the tail. This lift increases the nose-down pitching tendency.
Source: Dole, pg. 258
The decrease in the wing's lift causes a nose-down pitching moment. As the air above the wing goes above the horizontal stabilizer, the tail down force is decreased which also causes a nose-down moment. The aircraft is said to stall "conventionally."
T-tailed aircraft
Again assume that an aircraft is trimmed in straight and level flight.
Source: Dole, pg. 258
The T-tailed aircraft behaves similarly to the low-tailed aircraft at this point.
As the aircraft is slowed toward the stall, the handling characteristics are much the same as the low tailed aircraft, except that the high tail remains clear of the wing wake and retains its effectiveness. Continued speed reduction is, therefore, more efficient.
Source: Dole, pg. 258
The tail does not lose its effectiveness as the angle of attack is first increased.
The tail does not lose its effectiveness as the angle of attack is first increased.
At the stall two distinct things happen. The swept wing, high tailed airplane tends to suffer a marked nose-up pitch after the stall (this is explained in detail later), and the wing wake, which has now become low energy turbulent air, passes straight aft and immerses the T tail and makes it incapable of combating the nose-up pitch and so the aircraft continues to pitch up. The great reduction in lift and increase in drag cause a rapidly increasing descent path. Thus the AOA is increased, and the pitch-up problem is worsened. The airplane is thus well on its way to extreme angles of attack and deep stall.
The effect of wing sweep. In practice the whole wing does not stall at the same instant. Swept and tapered wings will tend to stall at the tips first because of the high wing loading at the tips. The boundary layer outflow also resulting from wing sweep slows the airflow and reduces the lift near the tips and further worsens the situation. This loss of lift outboard (and therefore aft) causes the center of pressure to move forward, which augments the pitch-up tendency.
Source: Dole, pg. 258
Because the swept wing stalls first at the tips, there is more lift inboard which is forward, causing a pitch up. The tail becomes immersed in the turbulent air and has less "grip" and is less effective combating this tendency, making the pitch up tendency worse. But this effect isn't true for all T-tail aircraft. You can engineer the camber of the stabilizer's airfoil to stall conventionally if you want, for example: Stalling a Tomahawk, courtesy TheCFIGuy. So the lesson here is to be wary of your airplane's stall characteristics. Even if you've stall that exact airplane before, any changes to loading and atmospheric conditions can surprise you.
4
How ground effect affects stall angle of attack
Most of us are taught early on that once the aircraft is within one-half wingspan height above the runway, we lose some drag because the wingtip vortices no longer wrap completely over the wing, reducing induced drag. And that is true. But it is only half the phenomenon. When you are in ground effect, you essentially have a different and more efficient wing. To understand a wing's performance In-Ground-Effect (IGE), you must first consider it Out-of-Ground-Effect (OGE).
Wing Performance Out-of-Ground-Effect (OGE)
You may have seen the vortices around your wingtips on a misty or rainy day and have an intuitive understanding. You have more pressure under the wing than over it, so the pressure below wants to wrap around to the top. Of course the wing is moving forward so this movement from bottom to top tends to trail aft. But why is this a big deal?
Wing tip vortices are formed when higher pressure air below a wing flows around the wing tips into the lower pressure region on top of a wing that is developing lift. The vortices are strongest at the tips and become weaker progressing toward the centerline of the aircraft.
Source: Dole, pg. 50
The downward force of the vortice not only subtracts from the upward force, it increases induced drag on the wing. It makes the wing less efficient. If only there was a way to remove the effect of the vortices. There is . . .
Wing Performance In-Ground-Effect (IGE)
Consider an aircraft with an infinitely long wing. This (infinite) wing has no wing tips and, consequently, no wing tip vortices. The absence of wing tip vortices on the infinite wing means that the up wash, in front of the wing, and the down wash, behind the wing, cancel each other out, and there is no net down wash behind the wing.
Source: Dole, pg. 50
You don't need an infinitely long wing, flying close enough to the ground will interrupt the vortices so they never get a change to reach the top of the wing. Since less of the aerodynamic force is pointed aft, you have less induced drag and more lift. To the pilot, the wing appears to have become more efficient because, really, it has!
IGE to OGE: Thrust Limitation
If you place the IGE and OGE coefficient of lift to AOA curves together, you will notice that the IGE curve moves to the left. There is research that indicates the CL-MAX (the peak of the curve) remains the same but there is also research that shows it is lower when IGE. More on that later, for now let's look at what happens to the thrust required to hold the AOA.
While the aerodynamic characteristics of the tail and fuselage are altered by ground effects, the principal effects due to proximity of the ground plane are the changes in the aerodynamic characteristics of the wing. As the wing encounters ground effect and is maintained at a constant lift coefficient, there is a reduction in the up wash, down wash, and the tip vortices. These effects are illustrated by the sketches of [the figure]. As a result of the reduced tip vortices, the wing in the presence of ground effect will behave as if it were of a greater aspect ratio. In other words, the induced velocities due to the tip (or trailing) vortices will be reduced and the wing will incur smaller values of induced drag coefficient, CDi, and induced angle of attack, αi, for any specific lift coefficient, CL.
Source: Hurt, pg. 379
Looking at the CL to AOA chart (on the left), you see that flying at a given AOA, you get more lift when IGE than OGE. The wing is more productive when in-ground-effect. But looking at the Thrust Required to Velocity chart (on the right), we see that for any given velocity, it takes more thrust to fly OGE.
The consequence for us in the balked landing situation is that we could find ourselves just barely climbing while in-ground-effect, and once we do climb out-of-ground-effect, we may not have enough thrust available to maintain airspeed.
IGE to OGE: Ground Effect Stall
After the 2011 crash of a test Gulfstream G650, we've heard a lot about the "ground effect stall" and how it was possible to stall the airplane while in ground effect (true) and that you could stall the airplane leaving ground effect (true, to a point).
Stalling In-Ground-Effect (IGE)
αstall — stall angle of attack
αstall,IGE — αstall in-ground-effect
αstall,OGE — stall out-of-ground-effect
The physical basis and qualitative effects of ground-effect on an airplane’s aerodynamics are well understood. The airflow around the airplane cannot have a vertical component at the ground plane (i.e., air cannot flow into or out of the ground), and so the streamlines around the airplane are altered from what they would be in free air. Specifically, the downwash produced by the wing trailing vortices is reduced by the proximity of the ground plane, which in turn reduces the induced angle of attack and induced drag, and increases the lift coefficient (CL) at a given α. These effects are sketched in [the figure]. The sketch illustrates that αstall,IGE is lower than αstall,OGE, and the sketch also suggests that the maximum CL achievable (CLMAX) in- and out- of ground-effect are the same. [ . . . ] However, other researchers have documented that CLMAX can decrease in ground-effect. [ . . . ] Given this disparity of evidence, the quantitative effects of ground-effect are likely unique to every airplane configuration, and must be evaluated either by test or analysis for each configuration.
Source: O'Callaghan, §VII
The study looked at CLMAX for an accident in a Gulfstream G650 and concluded:
CLMAX in-ground-effect is reduced compared to CLMAX out-of-ground-effect.
For more about this accident: Case Study: Gulfstream G650 N652GD.
Ground effect lowers induced drag and that in turn increases the lift coefficient for a given angle of attack. For some airplanes this also reduces the maximum coefficient of lift (CLMAX). The aircraft will stall at a lower angle of attack in ground effect. This is important for us to realize in a balked landing situation:
Notice how CL-max is lower when IGE in the chart from the G650 accident. Not all text books agree so we'll take a look at the more conservative view that CL-max remains the same for two hypothetical examples.
How slowly are you willing to fly after balking a landing? We quite often rely on our approach reference speed, commonly called V-REF, as the speed we know will keep us safe. It is usually based on 1.23 times the stall speed of the aircraft. But that stall speed is based on the stall AOA, which is determined while flying Out-of-Ground-Effect. So if you are balking a landing and are In-Ground-Effect, the VREF you think will keep you above the stall might not, since it is based being Out-of-Ground-Effect.
Let’s examine a hypothetical aircraft which just balked its landing while In-Ground-Effect.
- Suppose the CL-max while IGE is 10° and that equates to 115 KCAS in this situation. (Blue in the diagram.)
- Suppose also that the CL-max while OGE is 14° and that equates to 100 KCAS in this situation. (Red in the diagram.)
- In this situation, your VREF will probably be 123 KCAS (1.23 times the OGE stall speed).
- If you are just barely climbing IGE at 123 knots (the green line in the diagram), you might think you have a 23% margin above the stall and be tempted to pull back further on the stick.
- But until you leave ground effect, your true stall speed is 115 KCAS. If you pull back further than 115 KCAS while IGE, you will stall the aircraft.
The lesson here is to give your VREF a healthy margin when In-Ground-Effect.
Stalling Leaving Ground Effect
Can you be flying below the stall In-Ground-Effect and then stall simply by climbing Out-of-Ground-Effect? If you look at the CL curves while sitting in the safety and comfort of your desk, the answer appears to be no. But keep in mind all this is happening very fast and the transition from IGE to OGE may be a split second transition. It gets very dicey. Perhaps another hypothetical is in order. (Actually the same hypothetical, but just as the aircraft leaves ground effect.)
- Now let's say you maintain your calculated VREF of 123 KCAS all the way to the point your ground effect stops. (Point #1 on the chart.)
- You may argue that ground effect doesn't switch off from on and that's true. But it happens very fast. As it is happening, your CL drops from the IGE line to the OGE line and the airplane wants to stop climbing and you feel it sinking. (Point #2 on the chart.)
- So you pull back on the stick. If you do this just right, you will regain the original CL and perhaps a little more. But how well calibrated is your hand? All this happens very fast and the chance of overshooting your pull and ending up a CL-max while OGE is very high. (Point #3 on the chart.)
When you leave ground effect you have two performance "obstacles" to overcome: you will have a loss of lift coefficient and an increase in induced drag. As with our previous hypothetical, having a healthy margin above VREF is key. In either case, you must focus on airspeed before you can climb when balking a landing when In-Ground-Effect.
5
Proper stall recovery procedure
[Davies, pg. 92] In the [figure shown] the total drag curves have been plotted against speed for both a piston-engined and a jet aircraft. The two speed scales at the bottom show the relative changes, as a function of stall speed. . . . Notice the VIMD [the point of minimum drag] of 1.2 VS for the piston, this is typical. On the jet, however, VIMD is much higher at around 1.4 VS; this is typical of the latest jets, although earlier models were nearer 1.3 VS.
Flight below VIMD on a piston-engined aeroplane is quite well identified by the steepness of the drag curve; in flight, as well as on paper! But the relative flatness of the drag curve around VIMD for the jet does not produce any noticeable changes in flying qualities (other than a vague and irritating lack of speed stability), and all this is a bit of a trap.
All this means that when, for example, holding in the clean configuration in a jet transport at high altitude, with a speed in the neighborhood of 1.5 to 1.6 VS, care is necessary to avoid any decrease in airspeed; if this should occur the aeroplane will quietly slide up the back end of the drag curve. Although at higher lift coefficients associated with lower speeds some extra lift is produced, the drag increases faster than the lift increases, the L/D ratio deteriorates and the net result is a steepening of the flight path. So the aeroplane begins to sink. This sinking tendency can be salvaged in two ways:
- The nose can be put down to reduce incidence and allow the aeroplane to accelerate to a speed above VIMD, when steady flight conditions can be again established, but as this always involves a height loss it can rarely be used.
- The thrust can be increased to accelerate the aeroplane to a value above VIMD to establish steady flight conditions again. it is important to emphasize that the amount of thrust to accelerate the aeroplane is quite large.
So, if you suspect that this is what is occurring, don't play around with small increments of thrust hoping to sort things out without making it obvious that something has gone wrong — give it a handful. Fly the aeroplane with some determination and get it re-established in a steady flight condition as soon as possible.
Source: Davies, pg. 92
The text from D. P. Davies is a bit dated and I think you can substitute "straight wing" for "Piston-engined aircraft" and "swept wing" for "jet aircraft." But his bottom line is a good one: don't play around, give it all the power you can. Now adding what we know from the thirty years of history since this text was written, also give it a handful of pitch, you need to break the stall.
Your prime directive here is to get the wing comfortably flying again and that means you have to decrease the angle of attack. The best way to do that during a stall, or even an approach to a stall, is to decrease pitch. That is going to take a conscious push forward on a swept wing, T-tailed aircraft.
If you don't decrease pitch enough and the stick shaker becomes a pusher, do not override the pusher! Let it pitch the nose over and then try again to manually recover.
What if you are low to the ground you say? Well how much altitude is needed for the recovery? If you practice this realistically in the simulator it rarely takes more than 300'. If you are any lower than that you are no longer managing a stall, you are managing a very hard landing.
References
(Source material)
AC 120-109 Stall and Stick Pusher Training, 8/6/12, U.S. Department of Transportation
Davies, D. P., Handling the Big Jets, Civil Aviation Authority, Kingsway, London, 1985.
Dole, Charles E., Flight Theory and Aerodynamics, 1981, John Wiley & Sons, Inc, New York, NY, 1981.
Gulfstream G450 Aircraft Operating Manual (Historical), Revision 24, September 18, 2009.
NTSB Aircraft Accident Report, AAR-12/03, Crash During Experimental Test Flight, Gulfstream Aerospace Corporation GVI (G650), N652GD, Roswell, New Mexico, April 2, 2011
Please note: Gulfstream Aerospace Corporation has no affiliation or connection whatsoever with this website, and Gulfstream does not review, endorse, or approve any of the content included on the site. As a result, Gulfstream is not responsible or liable for your use of any materials or information obtained from this site.